Sponsored by 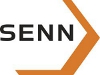
Introduction: From Peptides to Peptidomimetics
In recent years, an increasing number of peptides have been marketed as drugs, indicating their potential as new therapeutics.(1-3) Considerable progress has been made to improve the pharmacokinetic properties of peptides, and to develop convenient administration modes. Nevertheless, small molecule drugs still have a number of advantages such as a lower production cost, better permeability across biological membranes leading to good oral bioavailability and BBB passage. Therefore, much efforts have been made to obtain low molecular weight ligands for peptide receptors.(4-7) For such a peptidomimetic ligand, some authors use a strict definition of a peptide mimetic as a molecule that no longer contains amide bonds, whereas others use the term for any chemically modified peptide. (7,8) V. Hruby describes a peptidomimetic as a designed compound whose pharmacophoric structural elements mimic a peptide’s binding elements in 3D space, and which mimics the binding and agonist or antagonist activity of a natural peptide ligand.(9)
Many non-peptidic compounds acting at peptide receptors as agonists or antagonists have been discovered through screening strategies, followed by classical medicinal chemistry optimization. The structural relationship between many of these mimetics (they were also termed type II peptidomimetics or functional mimetics)(4) and the original peptide is often unclear, therefore they do contribute little to the development of a chemical strategy to design peptidomimetics starting from the parent peptide structure. (Figure 1)
Figure 1: Peptidomimetics discovered by screeningMany years of intense research efforts have resulted in the development of a hierarchical strategy to transform a bioactive peptide into analogs having gradually a less peptide character, and finally into a peptidomimetic. (7, 9-13) (Figure 2)
Figure 2: A hierarchical approach to peptidomimetic designAs a first step, information about the amino acid sequence which is essential for the bioactivity is generated using N- and C-terminal truncations, use of D-amino acids, non-coded amino acids, N-alkylations, .. etc. These methods maintain the peptide character of the derivative, and lead to shortened modified peptides.(7) Next, the topographic requirements for receptor interaction are investigated by a conformational constraining strategy. A global constraint of the peptide backbone is obtained by using different types of cyclization (11), using the “spatial screening” concept (12) or secondary structure mimetics; local constraints giving information about the side chain orientations (design in chi space) are obtained by using designed amino acids. (9,13)
In a further step the α-peptidic backbone can be modified by using various amide bond isosteres, C-substitutions such as azapeptides or chain extensions (homoamino acids), resulting in derivatives with partial peptide character, or pseudopeptides. The resulting information, together with data from conformational studies mainly by using NMR spectroscopy and molecular modeling, is used to generate a 3D pharmacophore model. The display of the pharmacophore elements on a scaffold results in the de novo design of a peptidomimetic. Evidently, the boundaries between these various classes are not sharply defined, a classification is often arbitrary. In the process a gradual shift from a peptide towards compounds with less peptidic character is resulting.
In a peptidomimetic, peptide pharmacophoric groups are displayed with a defined 3D orientation on a variety of scaffolds such as small heterocycles, including “privileged templates”. This “rational” approach requires considerable research efforts, and still represents a major challenge. Nevertheless, an increasing number of successful examples exist. One of the most studied peptides in this context is somatostatin (SRIF). It is an example which greatly contributed to the development of the hierarchical approach described in Figure 2, and where peptide modifications, pseudopeptide and peptidomimetic design have been applied with great success.(14-18)
Somatostatin
SRIF-14 is a cyclic tetradecapeptide which negatively regulates the secretion of a variety of hormones. (19) Five sub-type receptors were identified (sstr1-5). The use of SRIF-14 as a drug is limited to acute indications because of its short half life (2- 3 min). Systematic truncation studies identified the Phe-Trp-Lys-Thr tetrapeptide unit as the essential pharmacophore and a conformational constraining strategy resulted in the development of the cyclic octapeptide octreotide (Sandostatin®, half life 2 h) and of the cyclic Veber – Hirschman hexapeptide L-363,301.(15) (Figure 3) Octreotide is clinically approved for treatment of acromegaly, diarrhea due to VIPomas and carcinoid syndrome. It was at the basis for the development of various radiopharmaceuticals such as Octreoscan® and OctreoTher® for respectively diagnosis and peptide receptor radionuclide therapy of tumors. (19)
Figure 3: Somatostatin-14 and reduced size analogsExtensive structure-activity and conformational studies on these cyclic peptides indicated the presence of a β-II’ turn; stabilized by the D-configuration of Trp and a close proximity of the D-Trp and Lys side chains as essential features for bioactivity.
Modified Somatostatins
The cyclic scaffolds (Figure 3) served as inspiration for the development of a variety of modified and pseudopeptides (Figure 4): in the retro-inverso analog, the direction of the peptide bonds was reversed and the configuration of five residues was inverted. Its potency is comparable to somatostatin.(20)
Figure 4: Modified cyclic somatostatinsGilon applied a cyclization method using the backbone amide nitrogen to attach the linker, resulting in PTR 3046, with an IC50 of 67 nM for sstr5 and having a high enzymatic stability.(21)
From a library of 30 N-methylated analogs of the Veber-Hirschmann peptide, the triply N-methylated analog was identified. It was shown to have high affinity for sstr2 and sstr5 and had an oral bioavailability of ca 10% in rats, in contrast to the parent cyclopeptide which is not orally available. (22)
Hundreths of other analogs of octreotide and of the Merck cyclohexapeptide were prepared. All of them confirm that the D-Trp-Lys in a β-II’ turn conformation is an essential feature for bioactivity.(14)
Omatostatin Pseudopeptides and Peptidomimetics Based on the Main Chain Turn Conformation
Seebach used the propensity of 2/3-dipeptide and -dipeptide combinations to adopt turn stuctures, to develop derivatives with micro to nanomolar affinity, but with high selectivity. (Figure 5) The open chain β-tetrapeptide has a high selectivity for sstr4 (83 nM) , as is the cyclic analog, which is however less potent (4.6 µM).(23) A -dipeptide analog was selective for sstr5 (0.87 µM). (24) Interestingly an N-peptoid analog of the cyclo β-peptide was selective for sstr5, but with low potency (1.7 µM). (25)
Figure 5: Homo-peptides and peptoid as somatostatin mimicsA very often applied approach consisted of the use of scaffolds that were designed as mimics for the -turn conformation of the cyclic peptides, and display the important Trp and Lys pharmacophores with one or two additional aromatic groups. (Figure 6) The design of the somatostatin mimetic, based on the -D-glucose scaffold by Hirschmann was a landmark in this field. (26) Most of these mimetics showed µM affinities, only the cyclic thioether analog was a sstr5 selective ligand (87 nM).(32)
Figure 6: Somatostatin mimics based on a scaffold mimicking the Trp-Lys display on a -turn (26-32)The first potent somatostatin mimetics resulted from a 3D similarity search of the Merck compound collection using the Tyr-D-Trp-Lys motif. Biological testing yielded compound L-264,930 with 122 nM affinity for the human sstr2. (33) (Figure 7). Further screening of combinatorial libraries led to highly active and selective agonists for each of the five sstr’s. (Figure 7) Small modifications of the Merck compounds resulted in the conversion of agonists into antagonists. A change in the N-terminal substituent gave sstr2 selective antagonists. (34)(Figure 7)
Figure 7: subtype selective somatostatin agonists and antagonist.Somatostatin Peptidomimetics Based on the Trp Side Chain Conformation
Whereas most of the somatostatin mimetics described in Figures 5-7 were focussing on scaffolds mimicking the β-turn backbone conformation, our group has focused on the side chain conformation of the essential D-Trp-Lys residues. In the (2R,3S)β-MeTrp8 analogs of the Veber-Hirschmann cyclopeptide, evidence has been reported that the D-Trp sidechain adopts a trans conformation at 1. (35) Note that this (2R,3S)β-MeTrp is also present in mimetic L-779,976 .(Figure 7) Many conformational studies have shown that the indole ring of D-Trp8 is in close proximity to the Lys9 sidechain. (36)
Our group developed the cyclic Trp analog 4-amino-indolo[2,3-c]azepin-3-one (Aia) which constrains the side chain to a trans or gauche(+) conformation. (Figure 8) (37) Aia was first introduced into the linear tetrapeptide that is essential for somatostatin affinity. The change of the D-Trp residue in Ac-Phe-D-Trp-Lys-Thr-NH2 to an (R)-Aia led from an inactive tetrapeptide to an analog having nanomolar affinity for sstr4. This stimulated us to design somatostatin mimetics based an an (R)-Aia-Lys scaffold (Figure 9). Introducing an aromatic substituent at the N-terminus targets the receptor pocket occupied by Phe6/7/11 of the native ligand, as also proposed for various reported somatostatin mimetics. (Figures 6, 7)
Figure 8: Aia, a conformationally constrained TrpThese initial studies identified the N-phenylacetyl, benzyl amide as a potent mimetic with a preference for sst4 (3.3 nM) and sst5 (1.2 nM). (38) NMR studies in DMSO or chloroform did not show any indication for the presence of a β-turn conformation however. Nevertheless the high field shift of the lysine gamma CH2 protons at = 0.57 ppm indicates a close proximity to the Trp and Lys side chains as required for high receptor affinity. (35, 36) Subsequently, a series of analogs was prepared in which both the N- and C-terminal substituents were varied (39). This allowed us to identify analogs with low nM affinity for various subtypes of somatostatin receptors with different selectivities. The N-acetyl, phenylethyl amide analog (Figure 9, right) represents one of the most potent and selective sstr5 somatostatin ligands, whereas the piperidylurea ananalog (Figure 9, left) is an almost universal ligand. In a [35S]GTPS binding assay, both analogs were shown to be agonists. Docking of the N-phenylacetyl, benzyl amide lead compound into a homology modelled sstr4 revealed a perfect mimicry of the SRIF Phe6, Trp8 and Lys9 side chains.(40)
Figure 9: Aia-somatostatin analogsThe structural similarity of the somatostatin pharmacophore with that of urotensin (U-II, Figure 1) (41, 42) which contains a Trp-Lys sequence, stimulated us to test the Aia-containing somatostatin mimetics for U-II affinity. Interestingly, the Ac-(S)Aia-Lys-NHBn mimetic (the L-Aia epimer of the D-Aia somatostatin mimetics, Figure 9) which was inactive at the somatostatin receptors, showed a 1 µM affinity for the U-II receptor. (43) This indicates that further optimization of this scaffold using the same design principles might result in new U-II mimetics.
Conclusions
The conversion of a peptide into a peptide mimetic remains a very challenging task. The guidelines which have been developed over the years and the wealth of chemistries that has been applied, constitute a valuable help in the process, as was demonstrated for somatostatin. Other successful examples include the helix mimetic cyclic peptide and small molecule antagonists of the p53-HDM2 interaction(46,47) and the RGD-containing integrin ligands.(46) In future, the availability of X-ray crystallographic structures of peptide GPCR’s such as those for the CXCR4 (47) and neurotensin 1 receptor (48), will lead to a paradigm shift in the design of peptide mimetics, since structure-based design methods will become possible. However, the wealth of knowledge on peptide modifications that has been accumulated over the years will continue to be invaluable in this exercise.
References
- Albericio F., Kruger H.G., Therapeutic Peptides, Future Med. Chem.2012, 4, 1527-1531.
- Peptide Therapeutics Foundation, Development trends for peptide therapeutics 2010 Report Summary, http://www.peptidetherapeutics.org/PTF_report_summary_2010.pdf
- Craik D.J., Fairlie D.P., Liras S., Price D., The future of peptide-based drugs. Chem. Biol. Drug Des.2013, 81, 136-147.
- Freidinger R.M. Nonpeptidic ligands for peptide and protein receptors. Curr. Opin. Chem. Biol.1999, 3, 395-406.
- Blakeney J. S., Reid R. C., Le G. T., Fairlie D. P., Nonpeptidic ligands for peptide-activated G protein-coupled receptors. Chem. Rev.2007, 107, 2960-3041.
- Jones R.M., Boatman P.D., Semple G., Shin Y.-J., Tamura S.Y., Clinically validated peptides as templates for de novopeptidomimetic drug design at G-protein-coupled receptors. Curr. Opin. Pharmacol.2003, 3, 530-543.
- Adessi, C.; Soto, C. Converting a peptide into a drug: Strategies to improve stability and bioavailability. Curr. Med. Chem.2002, 9, 963-978.
- Moore G.J. Designing peptide mimetics. Trends Pharmacol. Sci.1994, 15, 124-129.
- Hruby, V. J.; Balse, P. M. Conformational and topographical considerations in designing agonist peptidomimetics from peptide leads. Curr. Med. Chem.2000, 7, 945-970.
- Marshall G. R. A hierarchical approach to peptidomimetic design. Tetrahedron1993, 49, 3547-3558.
- Kessler H. Peptide conformations. Conformation and biological activity of cyclic peptides. Angew. Chem. Int. Ed.1982, 21, 512-523.
- Kessler H., Gratias R., Hessler G., Gurrath M., Muller G. Conformation of cyclic peptides. Principle concepts and the design of selectivity and superactivity in bioactive sequences by spatial screening. Pure Appl. Chem.1996, 68, 1201-1205.
- Tourwé D., Maes V. Aspects of peptidomimetics other than turn mimetics. Chapter 3 in Peptide and protein design for biopharmaceutical applications. Ed. K. Jensen, Wiley,2009, pp 49-132.
- Janecka A., Zubrzycka M., Janecki T. Somatostatin analogs. J. . Pept. Res.2001, 58, 91-107.
- Ovadia O., Greenberg S., Laufer B., Gilon C., Hoffman A. ,Kessler H. Expert Opin. Drug Discov.2010, 5, 655-671.
- Wolkenberg S.E., Thut C.J. Recent progress in the discovery of selective, non-peptide ligands of somatostatin receptors. Curr. Opin. Drug Discov. & Devel. 2008, 11, 446-457.
- Pasternak A.; Pan Y.; Mosley R. T.; Rohrer S. P.; Birzin E. T.; Schaeffer J.; Patchett A. A.; Yang L. Diverse peptidyl privileged structures as potent somatostatin receptor ligands. Lett. Drug Des. & Discov.2004, 1, 121-125.
- Crider A.M., Witt K.A. Somatostatin sst4 ligands: chemistry and pharmacology. Mini-Revs. Med. Chem.2007, 7, 213-220.
- Weckbecker G., Lewis I., Albert R., Schmid H. A., Hoyer D., Bruns C. Opportunities in somatostatin research: Biological, chemical and therapeutic aspects. Nat. Rev. Drug Discovery2003, 2, 999-1017.
- Freidinger R. M. Design and synthesis of novel bioactive peptides and peptidomimetics. J. Med. Chem.2003, 46, 5553-5566.
- Gilon, C.; Huenges, M.; Mathä, B.; Gellerman, G.; Hornik, V.; Afargan, M.; Amitay, O.; Ziv, O.; Feller, E.; Gamliel, A.; Shohat, D.; Wanger, M.; Arad, O.; Kessler, H. A backbone-cylic, receptor 5-selective somatostatin analogue: Synthesis, bioactivity, and nuclear magnetic resonance conformational analysis. J. Med. Chem.1998, 41, 919-929.
- Biron E., Chatterjee J., Ovadia, O., Langenegger D., Brueggen J., Hoyer, D., Schmidt H.A., Jelinek, R., Gilon, C., Hoffman, A., Kessler H., Angew.Chem. Int. Ed.2008, 47, 2595-2599.
- Seebach D., Gardiner j; β-Peptidic peptidomimetics, Acc. Chem. Res.2008, 41, 1366-1375.
- Seebach, D.; Schaeffer, L.; Brenner, M.; Hoyer, D. Design and synthesis of gamma-dipeptide derivatives with submicromolar affinities for human somatostatin receptors. Angew. Chem. Int. Edit.2003, 42, 776-778.
- Caumes C., Hjelmgaard T., Roy O., Reynaud M., Servent D., Taillefumier C., Faure S. Synthesis and binding affinities for sst receptors of cyclic peptoid SRIF-mimetics. Med. Chem. Commun.2012, 3, 1531-1535.
- Hirschmann, R.; Nicolaou, K. C.; Pietranico, S.; Salvino, J.; Leahy, E. M.; Sprengeler, P. A.; Furst, G.; Smith, A. B.; Strader, C. D.; Cascieri, M. A.; Candelore, M. R.; Donaldson, C.; Vale, W.; Maechler, L. Nonpeptidal peptidomimetics with a beta-D-glucose scaffolding. A partial somatostatin agonist bearing a close structural relationship to a potent, selective substance P antagonist. J. Am. Chem. Soc.1992, 114, 9217-9218.
- Chagnault V., Lalot J., Murphy P. V. Synthesis of somatostatin mimetics based on 1-deoxynojirimycin. ChemMedChem.2008, 3, 1071-1076.
- Mowery, B. P.; Prasad, V.; Kenesky, C. S.; Angeles, A. R.; Taylor, L. L.; Feng, J. J.; Chen, W. L.; Lin, A.; Cheng, F. C.; Smith, A. B.; Hirschmann, R. Catechol: a minimal scaffold for non-peptide peptidomimetics of the i+1 and i+2 positions of the beta-turn of somatostatin. Org. Lett.2006, 8, 4397-4400.
- Papageorgiou, C.; Borer, X. A non-peptide ligand for the somatostatin receptor having a benzodiazepinone structure. Bioorg. Med. Chem. Lett. 1996, 6, 267-272.
- Damour, D.; Herman, F.; Labaudinière, R.; Pantel, G.; Vuilhorgne, M.; Mignani, S. Synthesis of novel proline and gamma-lactam derivatives as non-peptide mimics of Somatostatin/Sandostatin® . Tetrahedron1999, 55, 10135-10154.
- Chianelli, D.; Kim, Y. C.; Lvovskiy, D.; Webb, T. R. Application of a novel design paradigm to generate general nonpeptide combinatorial scaffolds mimicking beta turns: Synthesis of ligands for somatostatin receptors. Bioorg. Med. Chem.2003, 11, 5059-5068.
- Souers, A. J.; Virgilio, A. A.; Rosenquist, A.; Fenuik, W.; Ellman, J. A. Identification of a potent heterocyclic ligand to somatostatin receptor subtype 5 by the synthesis and screening of beta-turn mimetic libraries. J. Am. Chem. Soc.1999, 121, 1817-1825.
- Rohrer, S. P.; Birzin, E. T.; Mosley, R. T.; Berk, S. C.; Hutchins, S. M.; Shen, D. M.; Xiong, Y. S.; Hayes, E. C.; Parmar, R. M.; Foor, F.; Mitra, S. W.; Degrado, S. J.; Shu, M.; Klopp, J. M.; Cai, S. J.; Blake, A.; Chan, W. W. S.; Pasternak, A.; Yang, L. H.; Patchett, A. A.; Smith, R. G.; Chapman, K. T.; Schaeffer, J. M. Rapid identification of subtype-selective agonists of the somatostatin receptor through combinatorial chemistry. Science1998, 282, 737-740.
- Hay, B. A.; Cole, B. M.; DiCapua, F.; Kirk, G. W.; Murray, M. C.; Nardone, R. A.; Pelletier, D. J.; Ricketts, A. P.; Robertson, A. S.; Siegel, T. W. Small molecule somatostatin receptor subtype-2 antagonists. Bioorg. Med. Chem. Lett.2001, 11, 2731-2734.
- He, Y.-B., Huang Z., Raynor K., Reisine T., Goodman M. Synthesis and conformations of somatostatin-related cyclic hexapeptides incorporating specific α- and β-methylated residues. J. Am. Chem. Soc.1993, 115, 8066-8072.
- Grace, C.R.R., Erchegyi J., Koerber S.C., Reubi J.C., Rivier J., Riek R. Novel sst2-selective somatostatin agonists. Three-dimensional consensus structure by NMR. J. Med. Chem.2006, 49, 4487-4496.
- Pulka K., Feytens D., Van den Eynde I., Kosson P., MisickaA., Lipkowski A., Tourwé D., Synthesis of 4-amino-3-oxo-tetrahydroazepino[3,4-b]indoles: new conformationally constrained Trp analogues.Tetrah. 2007, 63, 1459-1466.
- Feytens D., Cescato R., Reubi J.C., Tourwé D., A new sst4/5-selective somatostatin peptidomimetic based on a constrained Trp scaffold. J. Med. Chem.2007; 50, 3397 – 3401.
- Feytens D., De Vlaeminck M., Cescato R., Tourwé D., Reubi J. C. Highly potent 4-amino-indolo[2,3-c]azepin-3-one-containing somatostatin mimetics with a range of sst receptor selectivities. J. Med. Chem. 2009, 52, 95-104. Correction in J. Med. Chem.2010, 53, 921-921.
- Liu Z., Crider A.M., Ansbro D., Hayes C., Kontoyianni M., A structure-based approach to understanding somatostatin receptor-4 agonism (sst4). J. Chem. Inf. Model.2012, 52, 171-186.
- Grieco, P.; Carotenuto, A.; Campiglia, P.; Marinelli, L.; Lama, T.; Patacchini, R.; Santicioli, P.; Maggi, C. A.; Rovero, P.; Novellino, E. Urotensin-II receptor ligands. From agonist to antagonist activity. J. Med. Chem.2005, 48, 7290-7297.
- Carotenuto A., Grieco P., Novellino E., Rovero P. Urotensin-II receptor peptide agonists. Med. Res. Reviews, 2004, 24, 577-588.
- Chatenet D., Touwé D, unpublished
- Haridas V. From peptides to non-peptide alpha-helix inducers and mimetics. Eur. J. Org. Chem.2009, 5112-5128.
- Murray J.K., Gellman S.H. Targeting protein-protein interactions: lessons from p53/MDM2. Biopolym. Pept. Sci.2007, 88, 657-686.
- Meyer A., Auemheimer J., Modlinger A., Kessler H. Targetting RGD recognizing integrins: drug development, biomaterial research, tumor imaging and targeting. Curr. Pharm. Design2006, 12, 2723-2747.
- Wu B., Chien E.Y.T., Mol C.D., Fenalti G., Liu W., Katrich V., Abagyan R., Brooun P., Bi F.C., Hamel D.J., Kuhn P., Handel T.M., Cherezov V., Stevens R.C. Structures of the CXCR4 chemokine GPCR with small-molecule and cyclic peptide antagonists. Science2010, 330, 1066-1071.
- White J.F., Noinaj N., Shibata Y., Love J., Kloss B., Xu F., Gvozdenovic-Jeremic J., Shah P., Shiloach J., Tate C.G., Grisshammer R. Structure of the agonist-bound neurotensin receptor. Nature 2012, 490, 508-513.