Introduction
Containers (also known as bags) are used to store final drug products, active pharmaceutical ingredients (APIs), and starting reagents and process intermediates used during the manufacturing of either the drug product itself or ingredients in that drug product. Additionally, sterile bags can be used in the manufacturing of biopharmaceuticals, either to store media and associated process solutions or as a bio-reaction vessel. It is common for such bags to be constructed from multi-layered polymeric films, where the film’s construction (number of layers, thickness of layers) and the choice of the plastics used in the layers are dictated by functional performance requirements for the bags. Sterile bags are typically rendered sterile via gamma irradiation. Common plastics used in such multilayered films include ethylene vinyl acetate (EVA) and polyethylene (PE), as they provide a relatively inert fluid contact layer and are relatively inexpensive. Nevertheless, extractables from EVA and PE materials are well documented [1-10] and it has been established that the levels of extractable low molecular weight acids in plastic films are increased when such films are sterilized by irradiation [6-10].
Data published concerning the migration of organic extractables from such multi-layered films used in disposable manufacturing process containers provide insight into the kinetic nature of the release of such low molecular weight acids [11]. In the current manuscript, these migration data are considered in the context of those factors which effect the migration of these types of extractables and in terms of the ability of a mathematical migration model to mimic the observed migration profiles.
Experimental
Test Articles
The experiments whose results are considered in this manuscript are described in greater detail in reference 11. In general terms, two process containers, manufactured from multi-layered plastic films, were examined for their extractables characteristics. As indicated in the reference, the film for the first container type (C1) was a four-layer composite consisting of ultra low-density polyethylene (ULDPE) as the solution contact inner layer, a polyethylene vinyl alcohol copolymer (EVOH) as the gas barrier layer, a polyethylene vinyl acetate (EVA) core layer, and an ULDPE outer skin layer. Although the thicknesses of the individual layers were not indicated, the total film thickness was 0.25mm. The film for the second container type (C2) was a three-layer composite with EVA as the solution contact layer, EVOH as the gas barrier layer, and linear low-density polyethylene (LLDPE) as the outer skin layer. The total thickness of this film was 0.34mm. Both polyethylene layers will be designated as PE in the remainder of the text.
Extraction
The data considered from this study are derived from one of the three extraction sequences described in reference 11. Specifically, 5 liter-sized containers, both un-irradiated and after irradiation at a dose of greater than 45kGy, were filled with 800mL of high purity water as the extracting solvent. Although other solvents were utilized, water is the most relevant for considering the migration of highly soluble low molecular weight organic acids. The filled containers were positioned in such a way that the contact surface area (solution to container) was 2cm2/mL and so that aliquots of the fill solution could be removed for testing. Filled units were stored at either room temperature or 45°C for up to 120 days. At days 1, 7, 14, 30, 60, 90, and 120 of storage, aliquots of the fill solution were removed from the containers and tested. Multiple replicates of the irradiated bags and a single replicate of the un-irradiated bags were extracted and tested.
Extract Analysis
The process, procedures, and methods used to analyze the fill solution extracts are described in reference 11. In general, the fill solutions were analyzed for total organic carbon (TOC) via appropriate instruments and methodologies. The levels of organic acids in the retrieved fill solutions were measured using ion chromatography (IC), performed using conventional columns, mobile phase gradients, and detection techniques. While the IC analysis considered analytes other than acetic acid, acetic acid is the predominant extracted organic acid and thus was specifically examined in this study. Thus data for other acids are not summarized herein. Although the fill solutions were also tested for organic extractables by reversed phase high performance liquid chromatography (RP-HPLC), these results are not relevant to the purpose of this manuscript and thus are not reported herein.
Results and Discussion
Analysis of Migration Profiles
The nature of the test articles and the conditions under which the study was conducted provide the opportunity to examine the kinetic aspects of the leaching of acetic acid. Given that the primary source of the low molecular weight organic acid extractables is expected to be the film’s EVA layers, the difference in structure between the two films provides an opportunity to consider structural aspects of extractables migration. Furthermore, as irradiation produces acetic acid, the use of irradiated and non-irradiated containers provides a means of assessing how the extractable’s total available pool (TAP) affects extractables accumulation levels. Finally, comparison of migration profiles, TOC versus organic acids, may provide insights into the nature of the material’s extractables. The TOC and acetic acid levels in the fill solutions as a function of storage time are summarized in Table 1. Trend plots of the concentrations of extracted substances versus storage time are shown in Figures 1 through 6.
Table 1. Concentrations of TOC and Acetic Acid in the Water Extracts. Data from reference 11
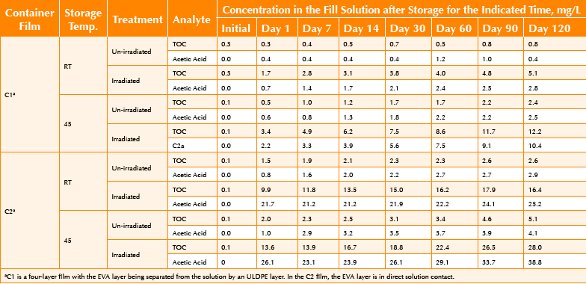
Figure 1. Effect of Irradiation on the Acetic Acid Migration Profiles. The upper profile is for container C1, leached at 45°C, and the lower profile is for container C2, leached at RT. The clear and obvious effect of irradiation is to increase the total pool of extractable acetic acid.It is intuitive to suspect that there will be major differences in the migration profiles as a function of material irradiation and the structure of the two container films. One anticipates that irradiation will increase the total pool of acetic acid; this effect is clearly illustrated in the increased equilibrium concentration in the migration plots of the irradiated versus the un-irradiated materials (Figure 1). Additionally, one anticipates that the migration will occur more quickly in material C2 than in material C1 given that material C2 has the EVA layer in direct solution contact (little or no migration pathway) and material C1 has the EVA “buried” in the film structure (specifically behind ULDPE and EVOH layers). As shown in Figure 2, the release of acetic acid from material C2 is virtually instantaneous (especially for the irradiated films), while the acetic acid release from material C1 is slower.
In addition to these obvious trends, the migration profiles contain more subtle traits that suggest certain complexities in the migration process. For example, consider the migration behavior shown by the C1 film, Figure 3. Addressing the irradiated film, the migration profiles have two distinct features. Firstly, the release of acetic acid at 45°C occurs quite rapidly and a level of 2mg/L of acetic acid is achieved after only 1 day of extraction. This length of time is too short for significant acetic acid to have migrated from the EVA layer and thus represents acetic acid that is more readily available than is the acetic acid associated with the EVA. Secondly, there is a considerable difference in the extraction profiles at the two temperatures. Although it is clear that the migration occurs slower at ambient temperature, the divergence of the profiles, ambient temperature versus 45°C is noteworthy. If the acetic acid were coming from a single source, such as the EVA, the ambient temperature profile would be less steep than the 45° profile but would eventually achieve the same equilibrium level as was achieved at 45°C, as the total pool of acetic acid is the same at both temperatures. Thus instead of diverging, the profiles at the two temperatures should converge. This divergence suggests that the acetic acid extracted at levels up to 2mg/L and that acetic level extracted thereafter arise from two different sources, a readily available source (contributing up to 2mg/L from a close proximity layer such as the solution contact LDPE) and a less readily available source (quantities greater than 2mg/L from a remote source such as the “buried” EVA layer). The plateau achieved at roughly 2mg/L at ambient temperature reflects primarily the readily available acetic acid; only at relatively long extraction times does the extraction profile suggest a second source of acetic acid.
Figure 2. Comparision of Migration Profiles as a Function of the Film Structure. In film C1, the primary source of acetic acid (the EVA layer) is “buried” in the film structure whereas the EVA layer in film C2 is the solution contact layer. While the acetic acid levels in the C2 film almost immediately reach their equilibrium value (because their migration pathway is small), there is a considerable lag in the migration profile of the C2 film as the acetic acid must migrate through both EVOH and ULDPE layers before it is released to the fill solution. The upper migration profile is for irradiated films, extracted at 45°C, and the lower profile is for the unirradiated films, also extracted at 45°C.The same behavior is exhibited for the non-irradiated C1 material. At 45°C, an acetic acid level of approximately 0.5mg/L is achieved after one day of storage and the acetic acid levels continues to increase thereafter. Roughly the same level of acetic acid (0.5mg/L) is attained at ambient temperature and this level does not increase appreciably with time. Again, this behavior is consistent with two acetic acid sources: one source whose migration pathway is short (immediate release of acetic acid) and the second whose migration pathway is longer (asymptotic release of acetic acid at levels above 0.5mg/L).
Figure 3. Migration Profile, Acetic Acid from Container C1 (“buried” EVA). The upper migration profile is for the irradiated material and the profile at the bottom is for the un-irradiated material. The divergence of the migration profiles, ambient temperature versus 45°C, occurs for both the irradiated and un-irradiated films. This divergence suggests that the film has two sources of acetic acid, one source which has a small diffusion pathway (accounting for the “immediate” release of acetic acid) and a second source which has a considerable diffusion pathway (accounting for the longer time, delayed release of acetic acid).
Figure 4. Migration Profile, Acetic Acid from Container C2. The upper migration profile is for the irradiated material and the profile at the bottom is for the un-irradiated material. The migration profiles for the irradiated film contain a discontinuity at approximately 20 days. Up to 20 days, the acetic acid concentration is roughly constant and temperature-independent, consistent with its source being the solution contact layer (EVA). After 20 days, the levels of acetic acid increase, roughly in proportion to the contact temperature. This suggests that the additional acetic acid is derived from a second, more remote source. A similar effect is refl ected in a divergence of the migrations profiles as a function of temperature for the un-irradiated materials.The migration profiles for the C2 material also exhibit a discontinuity but in this case the discontinuity is the inverse of that experienced by C1 and occurs at later extraction times (Figure 4). Considering the irradiated film, the level of acetic acid in the extraction solutions are roughly constant and independent of temperature through roughly 40 days of storage, reflecting the rapid release of readily available acetic acid. However, after roughly 20 days at 45° (approximately 60 days at ambient temperature), the profile exhibits a steady increase in acetic acid levels at both temperatures, consistent with a diffusion-controlled migration process. This behavior indicates that there is a secondary source of acetic acid in the C2 film. After the readily available acetic acid pool in the EVA is depleted, additionally acetic acid migrates from this second source. It takes this additional acetic acid approximately 40 days to transverse the film structure and reach the film/solution interface, where it is then leached into the fill solution.
Figure 5. Comparison of Migration Profiles, Total Organic Carbon (TOC) as a Surrogate for all Organic Extractables and Acetic Acid. The migration profiles are for film C1 at 45°C. The upper profile is for the irradiated material and the lower chromatogram is for the un-irradiated material. The profiles for TOC and acetic acid track each other well, establishing that the organic extractables behave similarly to acetic acid in terms of their migration properties.
Figure 6. Comparison of Migration Profiles, Total Organic Carbon (TOC) as a Surrogate for all Organic Extractables and Acetic Acid. The migration profiles are for film C2 at 45°C. The upper profile is for the irradiated material and the lower chromatogram is for the un-irradiated material. The profiles for TOC and acetic acid track each other well, establishing that the organic extractables behave similarly to acetic acid in terms of their migration properties.The mechanism behind the migration trends, acetic acid being sourced from two distinct sources, is clarified by the nature of the migration profies and their correlation to the film’s structures. In both the case of C1 and C2, the predominant source of acetic acid is the EVA layer. Thus in C1, with EVA “buried” in the film structure, the larger release of acetic acid occurs only after the acetic acid has migrated through the film layers that separate the EVA from the fill solution. Alternatively, the larger release of acetic acid in film C2 occurs immediately as the EVA is the solution contact layer in this film. The lesser release of acetic acid at early times in C1 and at later times in C2 is attributed to the solution contact layer of C1 and a “buried” layer in C2. Examination of the structures of C1 and C2 reveal that PE is the layer that meets these two criteria and it is concluded that the PE is the source of the secondary acetic acid. This is consistent with reports that low molecular weight organic acids are generated from PE via irradiation (for example, refs. 6–10).
Figure 7. Computer-Generated Migration Model of the Irradiated C1 Material. This profile was generated for the four layered film consistent with the conditions listed in the text. The computer-generated migration model has the same essential properties as the illustrated experimental profile, thereby supporting the hypothesis that the acetic acid is distributed primarily between the PE and EVA layers.Although acetic acid is the predominant extractable associated with both test materials, it is not the only extractable as it does not fully reconcile the TOC levels in the extracts. While the levels of acetic acid and TOC in Table 1 appear to be comparable, this is misleading as the carbon fraction of acetic acid is 0.4. Thus, for example, when the TOC and the acetic acid levels are the same, the acetic acid only accounts for 40% of the TOC. This situation notwithstanding, migration profiles for TOC and acetic acid can be compared to establish the migration characteristics of the other organic extractables. As shown in Figures 5 and 6, the migration profiles for the TOC and acetic acid track one another, suggesting that the unidentified organic extractables behave similarly to acetic acid in terms of their migration properties. This is not to say that the remaining extractables are structurally similar to acetic acid; in fact, the IC analyses clearly indicate that no other low molecular weight organic acid was present in the water extracts at levels comparable to acetic acid. Rather, these additional extractables are similar to acetic acid in terms of its high aqueous solubility, its defined total pool in the test materials, and its distribution in the test materials.
Figure 8. Computer-Generated Migration Model of the Irradiated C2 Material. This profile was generated for the three layered film consistent with the conditions indicated in the text. The computer-generated migration model has the same essential properties as the experimental profile, thereby supporting the hypothesis that the acetic acid is distributed primarily between the PE and EVA layers.Migration Modeling
The use of computer-based mathematical models to simulate and predict the migration of packaging components into packaged products is well-established in the food industry [12-16]. In fact, the use of mathematical migration models to establish the compatibility of food and its packaging is well-accepted and commonly utilized within the global regulatory community [17-19]. Although less frequently employed in pharmaceutical applications, the fundamental scientific principles that are the foundation of the mathematical models are equally valid for both food and pharmaceutical situations.
In the previous discussion of the migration profiles, we proposed essentially a compartmental mechanism for acetic acid migration, based on an empirical analysis of the available data. If that mechanism is valid, it should be that (a) the mechanism can be mathematically modeled and (b) the mathematical model should exhibit the same trends as were observed with the experimental data.
Because this manuscript utilizes data that were obtained from the literature (as opposed to from the author’s own laboratories), critical inputs needed for quantitative computerized models are unknown and unavailable. For example, it has been proposed that the films have two sources of acetic acid, the EVA layer and the PE layer. While the total pool of acetic acid in the films can be inferred from the data, the distribution of the acetic acid in the film’s layers cannot be established. Thus, mathematical modeling cannot generate an exact quantitative match to the experimental data. Nevertheless, mathematical models based on certain simplifying assumptions should produce calculated migration profiles that have the same general qualitative features of the experimental migration profiles.
To this end, commercially available migration modeling software [20] was used to generate qualitative migration profiles for acetic acid in the irradiated films. The computer-generated migration profiles were based on a film structure that assigned an appropriate thickness to the individual layers and distributed the total pool of acetic acid primarily between the EVA and PE layers. Ultimately, however, the models that most effectively mimicked the actual experimental profiles were those that assigned a small portion of the acetic acid to the EVOH layer. Specifically, C1 was modeled as a film that was predominately PE (in terms of relative thickness of the individual layers) and with an acetic acid distribution between the layers of approximately 1:1:5:1 (PE [solution contact]: EVOH:EVA:PE). C2 was also modeled as a film that was predominately PE (in terms of relative thickness of the individual layers) and with an acetic acid distribution between the layers of 14:1:4 (EVA [solution contact]: EVOH:PE).
Figures 7 and 8 are the computer-generated migration models for the irradiated C1 and C2 respectively. Their points of comparison are Figure 3 for C1 and Figure 4 for C2. In general, the experimental and the computer-generated profiles are similar. Considering C1 specifically, the experimental and computer-generated profiles both illustrate a rapid, largely temperature-independent release of acetic acid from the PE solution contact layer, followed by a slower release of acetic acid from the “buried” EVA layer. The experimental and computer-generated profiles mirror one another in establishing that the levels achieved at 25°C reflect the depletion of the surface layer’s acetic acid pool followed by the much slower (versus 45°C) release of acetic acid from the EVA
Considering C2, both the computer-generated profiles effectively mimic the experimental data. The computer-generated profile at 45°C mirrors the immediate release of acetic acid, followed by a period of approximately 10 days with no additional acetic acid build-up (lag of acetic acid coming from the “shielded” contact PE layer), further followed by the release for acetic acid that has migrated from the PE layer. Once again, the 25°C and 45° models agree in regard to the attainment of the initial asymptotic level of acetic acid as the same level is reached at both temperatures (albeit more slowly at the lower temperature).
Conclusions
Published data concerning the migration of extractables from two multi-layered films (PE, EVA, and EVOH layers) used in bio-processing solution bags have been examined with the intent of elucidating the migration mechanism. Because the films have different layer patterns, and because the films were tested before and after irradiation, the effect of film structure and irradiation on the migration of extractables, especially acetic acid, could be established.
The migration of acetic acid was affected by both irradiation and film structure. Irradiation affected the total pool of the extractables, especially volatile organic acids such as acetic acid. Film structure affected the shape of the migration profile. The data suggested that while EVA was the primary source of the extractable acetic acid, the PE was also a meaningful source of this extractable. Nevertheless, the shapes of the migration models indicate that the EVOH layer also contributes EVA to the extraction solution.
The hypotheses related to the migration mechanism were confirmed by the use of mathematical migration modeling software. Mathematically derived migration profiles closely fit the experimental profiles, confirming that the hypotheses around the migration profiles were viable.
Although the extractables profile of the films included compounds other than acetic acid, these compounds were not quantified so as to produce individual migration profiles. Nevertheless, the migration profiles for total organic extractables, as reflected in TOC, mirrored the migration profi les of acetic acid. Thus the unspecified extractables behave similarity to acetic acid with respect to the effects of irradiation and film structure on their migration behavior.
References
- A.D. Schwope; D. Till; D.J.Ehnholt; K.R. Sidman; R.H. Whelan; P.S. Schwartz; and R.C. Reid. Migration of Irganox 1010 from ethylene-vinyl acetate films to food and food-simulating liquids. Food & Chem. Toxicol. 1987; 25(4): 327-330
- Q. Xu; L.A. Trissel; and M.R. Davis. Compatibility of paclitaxel in 5% glucose and 0.9% sodium chloride injections with EVA minibags. Aust. J. Hosp. Pharm. 1998; 28(3): 156-159.
- D.R. Jenke; D. Zietlow; M.J. Garber; S. Sadain; D. Reiber; and W. Terbush. Accumulation of organic compounds leached from plastic materials in biopharmaceutical process containers. PDA J. Pharm. Sci. Tech. 2007; 61(4): 286-302.
- D. Jenke; D. Zietlow; and S. Sadain. Leaching of organic acids from irradiated EVA plastic as a function of solution pH and polarity. PDA J. Pharm. Sci. Tech. 2004; 58(1): 24-31.
- H. Chen; Y. Wu; X. Fu; and Z. Xia. Direct determination of acetophenone in ethylene vinyl acetate plastic by microwave assisted solid headspace-gas chromatography. Fenxi Huaxue. 2012; 40(6): 904-908.
- K. Azuma; T. Hirata; H. Tsunoda; T. Ishitani; and Y. Tanaka. Identification of volatiles from low density polyethylene film irradiated with an electron beam. Agric. Biol. Chem. 1983; 47(4): 855-860.
- K. Azuma; Y. Tanaka; H. Tsunoda; T. Hirata; and T. Ishitani. Eff ects of film variety on the amounts of carboxylic acids from electron beam irradiated polyethylene film. Agric. Biol. Chem. 1984; 48(8): 2003-2008.
- R. Buchalla; C. Boess; and K.W. Bogl. Characterization of volatile radiolysis products in radiation-sterilized plastics by thermal desorption-gas chromatography-mass spectrometry; screening of six medical plastics. Rad. Phys. Chem. 1999; 56(3): 353-367.
- R. Buchalla; C. Boess; and K.W. Bogl. Analysis of volatile radiolysis products in gamma-irradiated LDPE and polypropylene fi lms by thermal desorption-gas chromatography-mass spectrometry. Appl. Rad. Isotopes 2000; 52(2): 251-269.
- S. Chytiri; A.E. Goulas; A. Badeka; K.A. Riganakos; and M.G. Kontominas. Volatile and non-volatile radiolysis products in irradiated multilayer coextruded food-packaging films containing a buried layer of recycled low-density polyethylene. Food Add. & Contam. 2005; 22(12): 1264-1273.
- Extactables Testing Report, Millipore Corporation. PureFlex™ and SureFlex™Disposable Process Container Films. A White Paper (document TB2765ENOO Rev. A) published by the Millipore Corporation (Billerica, MA), 2009.
- T. Begley; L. Castle; A. Feigenbaum; R. Franz; K. Hinrichs; T. Lickly; P. Mercea; M. Milana; A. O'Brien; S. Rebre; R. Rijk; and O. Piringer. Evaluation of migration models that might be used in support of regulations for food-contact plastics. Food Add. & Contam. 2005; 22(1): 73-90.
- J. Brandsch; P. Mercea; M. Rüter; V. Tosa; and O. Piringer. Migration modelling as a tool for quality assurance of food packaging. Food Add. & Contam. 2002; 19(Supplement): 29-41.
- A. O’Brien and I. Cooper. Polymer additive migration to food—a direct comparison of experimental data and values calculated from migration models for polypropylene. Food Add. & Contam. 2001; 18: 343-355.
- J.H. Petersen; X.T. Trier; and B. Fabech. Mathematical modelling of migration: a suitable tool for the enforcement authorities? Food Add. & Contam. 2005; 22(10): 938–944.
- B. Roduit; C.H. Borgeat; S. Cavin; C. Fragniere; and V. Dudler. Application of Finite Element Analysis (FEA) for the simulation of release of additives from multilayer polymeric packaging structures. Food Add. & Contam. 2005; 22(10): 945-955.
- DFI. Ordonnance du Departement federal de l’interieur sur les materiaux et objets en matiere plastique. (OPla, RS 817.041.1). 2004. http://www. admin.ch/ch/f/rs/8/817.041.1.fr.pdf.
- European Commission Directive (EU) No. 10/2011 on plastic materials and articles intended to come into contact with food. Offi cial Journal of the European Union, 14 January 2011. L12/15.1.2011.
- EC. Applicability of generally recognized diff usion models for the estimation of specific migration is support of EU Directive 2002/72/EC. ERUR 24514 EN2010. 2010.
- AKTS AG. General description of the new SML software5. SML version 5.082. http://www.akts.com/sml/html.
Dr. Dennis Jenke is a Baxter Distinguished Scientist at Baxter Healthcare Corporation. He has published extensively in the areas of analytical chemistry, environmental science, and material/solution compatibility, serves as an expert reviewer for pharmaceutical and analytical journals, is a member of professional and standard-setting organizations whose charter is to establish best demonstrated practices for material/ solution compatibility, and is a frequent speaker on the subject of material/solution compatibility. He authored the book, Compatibility of Pharmaceutical Solutions and Contact Materials; Safety Considerations Associated with Extractables and Leachables.
Dr. Vishal Barge is a Research Scientist at Baxter, serving as a subject matter expert (SME) on topics related to analytical chemistry and extraxctables and leachables assessment.